Optogenetics: Therapeutic spark in neuropathic pain
DOI:
https://doi.org/10.17305/bjbms.2019.4114Keywords:
Neuropathic Pain, optogenetics, treatmentAbstract
Optogenetics is an emerging field, which uses light and molecular genetics to manipulate the activity of live cells by expressing light-sensitive proteins. With the discovery of bacteriorhodopsin, a light-sensitive bacterial protein, in 1971 Oesterhelt and Stoeckenius laid the pavement of optogenetics. However, the cross-integration of different disciplines is a little more than a decade old. The toolbox contains fluorescent sensors and optogenetic actuators that enable visualization of signaling events and manipulation of cellular activities, respectively. Neuropathic pain is pain caused either by damage or disease that affects the somatosensory system. The exact mechanism for neuropathic pain is not known, however proposed mechanisms include immune reactions, ion channel expressions, and inflammation. Current regimen for the disease provides about 50% relief for only 40–60% of patients. Recent in vivo and in vitro studies demonstrate the potential therapeutic applications of optogenetics by manipulating the activity of neurons. This review summarizes the basic concept, therapeutic applications for neuropathy, and potential of optogenetics to reach from bench to bedside in the near future.
Citations
Downloads
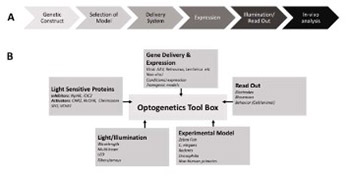
Downloads
Additional Files
Published
How to Cite
Accepted 2019-03-11
Published 2019-11-08