MicroRNA-126 enhances the biological function of endothelial progenitor cells under oxidative stress via PI3K/Akt/GSK3β and ERK1/2 signaling pathways
DOI:
https://doi.org/10.17305/bjbms.2019.4493Keywords:
Acute myocardial infarction, AMI, endothelial progenitor cells, EPC, PI3K/Akt/GSK3β pathway, ERK1/2Abstract
Endothelial progenitor cell (EPC) transplantation is a safe and effective method to treat acute myocardial infarction (AMI). However, oxidative stress leads to the death of a large number of EPCs in the early stage of transplantation, severely weakening the therapeutic effect. Previous studies demonstrated that microRNAs regulate the biological function of EPCs. The aim of the current study was to investigate the effect of microRNA on the biological function of EPCs under oxidative stress. Quantitative reverse transcription PCR was performed to detect the expression of miR-126, miR-508-5p, miR-150, and miR-16 in EPCs from rats, among which miR-126 showed a relatively higher expression. Treatment with H2O2 decreased miR-126 expression in EPCs in a dose-dependent manner. EPCs were further transfected with miR-126 mimics or inhibitors, followed by H2O2 treatment. Overexpression of miR-126 enhanced the proliferation, migration, and tube formation of H2O2-treated EPCs. MiR-126 overexpression also inhibited reactive oxygen species and malondialdehyde levels and enhanced superoxide dismutase levels, as well as increased angiopoietin (Ang)1 expression and decreased Ang2 expression in H2O2-treated EPCs. Moreover, miR-126 participated in the regulation of phosphatidylinositol 3-kinase (PI3K)/protein kinase B (Akt)/glycogen synthase kinase 3β (GSK3β) and extracellular signal-regulated kinase 1/2 (ERK1/2) signaling in EPCs, where both pathways were activated after miR-126 overexpression in H2O2-treated EPCs. Overall, we showed that miR-126 promoted the biological function of EPCs under H2O2-induced oxidative stress by activating the PI3K/Akt/GSK3β and ERK1/2 signaling pathway, which may serve as a new therapeutic approach to treat AMI.
Citations
Downloads
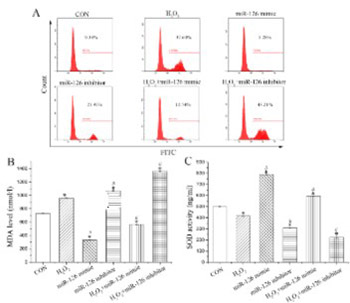
Downloads
Additional Files
Published
Issue
Section
Categories
How to Cite
Accepted 2019-12-26
Published 2021-02-01