ROS responsive polyethylenimine-based fluorinated polymers for enhanced transfection efficiency and lower cytotoxicity
DOI:
https://doi.org/10.17305/bjbms.2021.6704Keywords:
Fluorination, ROS-responsive, serum-resistant, polycation, transfectionAbstract
Cationic polymer polyethylenimine (PEI) plays a crucial role in gene delivery. However, high molecular weight PEI leads to higher efficient transfection efficacy and higher cytotoxicity while low molecular weight PEI exhibits lower transfection performance with lower toxicity. Therefore, effective chemical modification of PEI is required to enhance transfection activity and improve biocompatibility. Here, reactive oxygen species (ROS) responsive PEI-based fluorinated polymers (TKPF) with three degrees of fluorination (TKPF12.5%, TKPF25% and TKPF50%) were designed and synthesized by crosslinking low molecular weight PEI (PEI 1.8K) with a thioketal (TK) linker and then modifying heptafluorobutyric anhydride onto their surface. Such gene vectors exhibited the following features: (1) fluorination reduced the positive charge density and endowed hydrophobic and lipophobic characteristics to resist serum interactions; (2) The fluorophilic effect mediated efficient cellular uptake and endosomal escape; (3) ROS-responsive TK linker allowed the polyplex disassembly to decrease the cytotoxicity of the polycations and improve the release of payloads at specific sites. TKPFs attained superior transfection efficiency in multiple cell lines (293TN cells and B16F10 cells) in vitro and showed excellent biocompatibility. Notably, TKPFs also exhibited great serum resistance in gene delivery and TKPF50% transfected nearly 80% cells in the presence of 70% FBS. These results demonstrates that the fluorination and ROS responsiveness combined polycations are excellent gene-delivery vectors with serum-resistant capacity for further application.
Citations
Downloads
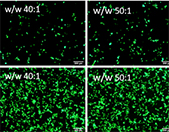
Downloads
Additional Files
Published
License
Copyright (c) 2022 Peng Hua, Donglin Yang, Ruie Chen, Peiqi Qiu, Meiwan Chen

This work is licensed under a Creative Commons Attribution 4.0 International License.
How to Cite
Accepted 2021-12-12
Published 2022-07-29