Astrocytes and human artificial blood-brain barrier models
DOI:
https://doi.org/10.17305/bjbms.2021.6943Keywords:
Astrocytes, blood-brain barrier, in vitro models, bioengineeringAbstract
The blood-brain barrier (BBB) functions as a highly selective border of endothelial cells, protecting the central nervous system from potentially harmful substances by selectively controlling the entry of cells and molecules, including components of the immune system. To study the BBB properties, find suitable therapies, and identify new drug targets, there is a need to develop representative in vitro BBB models. In this article, we describe the astrocyte roles in the BBB functioning and human in vitro BBB models.
Citations
Downloads
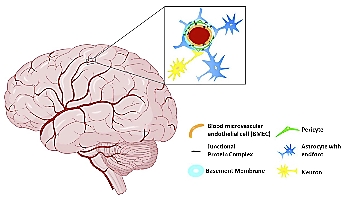
Downloads
Additional Files
Published
License
Copyright (c) 2022 Tanja Zidarič, Lidija Gradišnik, Tomaž Velnar

This work is licensed under a Creative Commons Attribution 4.0 International License.
How to Cite
Accepted 2022-03-09
Published 2022-09-16