LKB1 suppression promotes cardiomyocyte regeneration via LKB1-AMPK-YAP axis
DOI:
https://doi.org/10.17305/bjbms.2021.7225Keywords:
LKB1, cardiomyocyte proliferation, AMPK, YAPAbstract
This article has been corrected. Correction: https://www.bjbms.org/ojs/index.php/bjbms/article/view/12567
The regenerative potential of cardiomyocytes in adult mammals is limited. Previous studies reported that cardiomyocyte proliferation is suppressed by AMP-activated protein kinase (AMPK). The role of liver kinase B1 (LKB1), as the major upstream kinase for AMPK, on cardiomyocyte proliferation is unclear. In this study, we found that the LKB1 levels rapidly increased after birth. With loss- and gain-of-function study, our data demonstrated that LKB1 levels negatively correlate with cardiomyocyte proliferation. We next identified Yes-associated protein (YAP) as the downstream effector of LKB1 using high-throughput RNA sequencing. Our results also demonstrated that AMPK plays an essential role in Lkb1 knockdown-induced cardiomyocyte proliferation. Importantly, deactivated AMPK abolished the LKB1-mediated regulation of YAP nuclear translocation and cardiomyocyte proliferation. Thus, our findings suggested the role of LKB1-AMPK-YAP axis during cardiomyocyte proliferation, which could be used as a potential target for inducing cardiac regeneration after injury.
Citations
Downloads
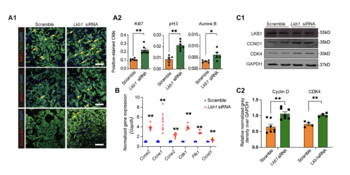
Downloads
Additional Files
Published
License
Copyright (c) 2022 Shuang Qu, Qiao Liao, Cheng Yu, Yue Chen, Han Luo, Xuewei Xia, Duofen He, Zaicheng Xu, Pedro A. Jose, Zhuxin Li, Wei Eric Wang, Qing Rex Lyu, Chunyu Zeng

This work is licensed under a Creative Commons Attribution 4.0 International License.
How to Cite
Accepted 2022-04-22
Published 2022-09-16