Mitoxantrone suppresses vascular smooth muscle cell (VSMC) proliferation and balloon injury-induced neointima formation: An in vitro and in vivo study
DOI:
https://doi.org/10.17305/bjbms.2017.2113Keywords:
Neointima formation, mitoxantrone, vascular smooth muscle cells, VSMCs, Type II topoisomerase, balloon injury model, proliferationAbstract
Neointima formation, which occurs after vascular injury due to vascular disease or interventions such as angioplasty and stent placement, is a complex process that involves multiple molecular and cellular mechanisms. The inhibition of neointima formation is vital to prevent restenosis of blood vessels. In the present study, we investigated whether the systemic administration of mitoxantrone can inhibit neointima formation, and evaluated the underlying mechanisms under in vitro and in vivo experimental conditions. In vitro, rat and human vascular smooth muscle cells (RVSMCs and HVSMCs) were stimulated with platelet-derived growth factor-BB (PDGF-BB) and treated with mitoxantrone or DMSO as a control. In vivo, 54 male Sprague-Dawley rats were subjected to carotid artery balloon injury and then intravenously administered with mitoxantrone. Cell proliferation was determined using the CCK-8 assay. Cell cycle analysis was performed using flow cytometry, and protein expression was analyzed by sodium dodecyl sulfate polyacrylamide gel electrophoresis. We used monoclonal mouse anti-bromodeoxyuridine (BrdU) antibody for the detection of BrdU and anti-Topoisomerase II antibody for staining Type II topoisomerase (Topo II), one week after the ballon injury. In both RVSMCs and HVSMCs, mitoxantrone treatment induced Topo II degradation, as well as suppressed DNA replication, cell cycle progression, and VSMC proliferation. A reduction in intimal hyperplasia, intimal-to-medial area ratio, and Topo II level was observed in mitoxantrone-treated rats, as compared to the control (saline) group. Overall, our results indicate that systemic administration of mitoxantrone can reduce neointimal hyperplasia and, thus, represents a suitable option for restenosis treatment.
Citations
Downloads
References
Orr AW, Hastings NE, Blackman BR, Wamhoff BR. Complex regulation and function of the inflammatory smooth muscle cell phenotype in atherosclerosis. J Vasc Res 2010;47(2):168-80. https://doi.org/10.1159/000250095.
Yu H, Payne TJ, Mohanty DK. Effects of slow, sustained, and rate-tunable nitric oxide donors on human aortic smooth muscle cells proliferation. Chem Biol Drug Des 2011;78(4):527-34. https://doi.org/10.1111/j.1747-0285.2011.01174.x.
Bharadwaj P, Chadha DS. Drug eluting stents: To evolve or dissolve? Med J Armed Forces India 2016;72(4):367-72. https://doi.org/10.1016/j.mjafi.2016.09.002.
Yurtkuran A, Tok M, Emel E. A clinical decision support system for femoral peripheral arterial disease treatment. Comput Math Methods Med 2013;2013:898041. https://doi.org/10.1155/2013/898041.
Yamasaki Y, Miyoshi K, Oda N, Watanabe M, Miyake H, Chan J, et al. Weekly dosing with the platelet-derived growth factor receptor tyrosine kinase inhibitor SU9518 significantly inhibits arterial stenosis. Circ Res 2001;88(6):630-6. https://doi.org/10.1161/01.RES.88.6.630.
Sachinidis A, Locher R, Vetter W, Tatje D, Hoppe J. Different effects of platelet-derived growth factor isoforms on rat vascular smooth muscle cells. J Biol Chem 1990;265(18):10238-43.
Leppänen O, Janjic N, Carlsson MA, Pietras K, Levin M, Vargeese C, et al. Intimal hyperplasia recurs after removal of PDGF-AB and-BB inhibition in the rat carotid artery injury model. Arterioscler Thromb Vasc Biol 2000;20(11):E89-95. https://doi.org/10.1161/01.ATV.20.11.e89.
Sherr CJ, Roberts JM. CDK inhibitors: Positive and negative regulators of G1-phase progression. Genes Dev 1999;13(12):1501-12. https://doi.org/10.1101/gad.13.12.1501.
Lefkovits J, Topol EJ. Pharmacological approaches for the prevention of restenosis after percutaneous coronary intervention. Prog Cardiovasc Dis 1997;40(2):141-58. https://doi.org/10.1016/S0033-0620(97)80006-0.
Fujimoto S, Ogawa M. Antitumor activity of mitoxantrone against murine experimental tumors: Comparative analysis against various antitumor antibiotics. Cancer Chemother Pharmacol 1982;8(2):157-62. https://doi.org/10.1007/BF00255476.
Holmes FA, Yap HY, Esparza L, Buzdar AU, Hortobagyi GN, Blumenschein GR. Mitoxantrone, cyclophosphamide, and 5-fluorouracil in the treatment of hormonally unresponsive metastatic breast cancer. Semin Oncol 1984;11(3 Suppl 1):28-31.
Velasquez WS, Lew D, Grogan TM, Spiridonidis CH, Balcerzak SP, Dakhil SR, et al. Combination of fludarabine and mitoxantrone in untreated stages III and IV low-grade lymphoma: S9501. J Clin Oncol 2003;21(10):1996-2003. https://doi.org/10.1200/JCO.2003.09.047.
Thomas X, Archimbaud E. Mitoxantrone in the treatment of acute myelogenous leukemia: A review. Hematol Cell Ther 1997;39(4):63-74.
https://doi.org/10.1007/s00282-997-0163-8.
Smith PJ, Morgan SA, Fox ME, Watson JV. Mitoxantrone-DNA binding and the induction of topoisomerase II associated DNA damage in multi-drug resistant small cell lung cancer cells. Biochem Pharmacol 1990;40(9):2069-78.
https://doi.org/10.1016/0006-2952(90)90237-F.
Feofanov A, Sharonov S, Kudelina I, Fleury F, Nabiev I. Localization and molecular interactions of mitoxantrone within living K562 cells as probed by confocal spectral imaging analysis. Biophys J 1997;73(6):3317-27. https://doi.org/10.1016/S0006-3495(97)78356-5.
Ehninger G, Schuler U, Proksch B, Zeller KP, Blanz J. Pharmacokinetics and metabolism of mitoxantrone. A review. Clin Pharmacokinet 1990;18(5):365-80. https://doi.org/10.2165/00003088-199018050-00003.
Ho CK, Law SL, Chiang H, Hsu ML, Wang CC, Wang SY. Inhibition of microtubule assembly is a possible mechanism of action of mitoxantrone. Biochem Biophys Res Commun 1991;180(1):118-23. https://doi.org/10.1016/S0006-291X(05)81263-X.
Golubovskaya VM, Ho B, Zheng M, Magis A, Ostrov D, Cance WG. Mitoxantrone targets the ATP-binding site of FAK, binds the FAK kinase domain and decreases FAK, Pyk-2, c-Src, and IGF-1R in vitro kinase activities. Anticancer Agents Med Chem 2013;13(4):546-54. https://doi.org/10.2174/1871520611313040003.
Wan X, Zhang W, Li L, Xie Y, Li W, Huang N. A new target for an old drug: Identifying mitoxantrone as a nanomolar inhibitor of PIM1 kinase via kinome-wide selectivity modeling. J Med Chem 2013;56(6):2619-29. https://doi.org/10.1021/jm400045y.
Bidaud-Meynard A, Arma D, Taouji S, Laguerre M, Dessolin J, Rosenbaum J, et al. A novel small-molecule screening strategy identifies mitoxantrone as a RhoGTPase inhibitor. Biochem J 2013;450(1):55-62. https://doi.org/10.1042/BJ20120572.
Dirsch O, Dahmen U, Gu YL, Ji Y, Karoussos IA, Wieneke H, et al. Preliminary investigation of mitoxantrone coating on stent-grafts to inhibit neointimal proliferation. J Endovasc Ther 2005;12(4):479-85. https://doi.org/10.1583/04-1444MR.1.
Liu Y, Li W, Ye C, Lin Y, Cheang TY, Wang M, et al. Gambogic acid induces G0/G1 cell cycle arrest and cell migration inhibition via suppressing PDGF receptor ß tyrosine phosphorylation and Rac1 activity in rat aortic smooth muscle cells. J Atheroscler Thromb 2010;17(9):901-13. https://doi.org/10.5551/jat.3491.
Wang M, Li W, Chang GQ, Ye CS, Ou JS, Li XX, et al. MicroRNA-21 regulates vascular smooth muscle cell function via targeting tropomyosin 1 in arteriosclerosis obliterans of lower extremities. Arterioscler Thromb Vasc Biol 2011;31(9):2044-53. https://doi.org/10.1161/ATVBAHA.111.229559.
Nyamekye I, Buonaccorsi G, McEwan J, MacRobert A, Bown S, Bishop C. Inhibition of intimal hyperplasia in balloon injured arteries with adjunctive phthalocyanine sensitised photodynamic therapy. Eur J Vasc Endovasc Surg 1996;11(1):19-28. https://doi.org/10.1016/S1078-5884(96)80130-4.
Gabeler EE, van Hillegersberg R, Statius van Eps RG, Sluiter W, Gussenhoven EJ, Mulder P, et al. A comparison of balloon injury models of endovascular lesions in rat arteries. BMC Cardiovasc Disord 2002;2:16. https://doi.org/10.1186/1471-2261-2-16.
Sollott SJ, Cheng L, Pauly RR, Jenkins GM, Monticone RE, Kuzuya M, et al. Taxol inhibits neointimal smooth muscle cell accumulation after angioplasty in the rat. J Clin Invest 1995;95(4):1869-76. https://doi.org/10.1172/JCI117867.
Stone GW, Moses JW, Ellis SG, Schofer J, Dawkins KD, Morice MC, et al. Safety and efficacy of sirolimus-and paclitaxel-eluting coronary stents. N Engl J Med 2007;356(10):998-1008. https://doi.org/10.1056/NEJMoa067193.
Weisz G, Leon MB, Holmes DR Jr, Kereiakes DJ, Popma JJ, Teirstein PS, et al. Five-year follow-up after sirolimus-eluting stent implantation results of the SIRIUS (Sirolimus-eluting stent in de-novo native coronary lesions) trial. J Am Coll Cardiol 2009;53(17):1488-97. https://doi.org/10.1016/j.jacc.2009.01.050.
Grube E, Silber S, Hauptmann KE, Mueller R, Buellesfeld L, Gerckens U, et al. TAXUS I: Six-and twelve-month results from a randomized, double-blind trial on a slow-release paclitaxel-eluting stent for de novo coronary lesions. Circulation 2003;107(1):38-42. https://doi.org/10.1161/01.CIR.0000047700.58683.A1.
Widmark A. New principles in the treatment of prostate cancer - The oncologist's view. Scand J Urol Nephrol Suppl 2003;212:23-7. https://doi.org/10.1080/03008880310006913.
Eilber FC, Rosen G, Forscher C, Nelson SD, Dorey F, Eilber FR. Recurrent gastrointestinal stromal sarcomas. Surg Oncol 2000;9(1):71-5.
https://doi.org/10.1016/S0960-7404(00)00026-8.
Jeffery DR, Herndon R. Review of mitoxantrone in the treatment of multiple sclerosis. Neurology 2004;63(12 Suppl 6):S19-24. https://doi.org/10.1212/WNL.63.12_suppl_6.S19.
Buerke M, Guckenbiehl M, Schwertz H, Buerke U, Hilker M, Platsch H, et al. Intramural delivery of sirolimus prevents vascular remodeling following balloon injury. Biochim Biophys Acta 2007;1774(1):5-15. https://doi.org/10.1016/j.bbapap.2006.04.018.
Kimura T, Morimoto T, Natsuaki M, Shiomi H, Igarashi K, Kadota K, et al. Comparison of everolimus-eluting and sirolimus-eluting coronary stents: 1-year outcomes from the randomized evaluation of sirolimus-eluting versus everolimus-eluting stent trial (RESET). Circulation 2012;126(10):1225-36. https://doi.org/10.1161/CIRCULATIONAHA.112.104059.
Waksman R, Pakala R, Baffour R, Seabron R, Hellinga D, Chan R, et al. In vivo comparison of a polymer-free biolimus A9-eluting stent with a biodegradable polymer-based biolimus A9 eluting stent and a bare metal stent in balloon denuded and radiated hypercholesterolemic rabbit iliac arteries. Catheter Cardiovasc Interv 2012;80(3):429-36. https://doi.org/10.1002/ccd.23407.
Piccolo R, Galasso G, Piscione F, Esposito G, Trimarco B, Dangas GD, et al. Meta-analysis of randomized trials comparing the effectiveness of different strategies for the treatment of drug-eluting stent restenosis. Am J Cardiol 2014;114(9):1339-46. https://doi.org/10.1016/j.amjcard.2014.07.069.
Gallo R, Padurean A, Jayaraman T, Marx S, Roque M, Adelman S, et al. Inhibition of intimal thickening after balloon angioplasty in porcine coronary arteries by targeting regulators of the cell cycle. Circulation 1999;99(16):2164-70. https://doi.org/10.1161/01.CIR.99.16.2164.
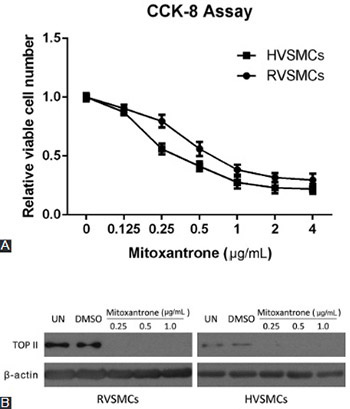
Downloads
Additional Files
Published
Issue
Section
Categories
How to Cite
Accepted 2017-05-09
Published 2017-11-20