MPTP-induced mouse model of Parkinson’s disease: A promising direction of therapeutic strategies
DOI:
https://doi.org/10.17305/bjbms.2020.5181Keywords:
Parkinson's disease, MPTP, C57BL mouse, MPTP-induced PD mouseAbstract
Among the popular animal models of Parkinson’s disease (PD) commonly used in research are those that employ neurotoxins, especially 1-methyl- 4-phenyl-1, 2, 3, 6-tetrahydropyridine (MPTP). This neurotoxin exerts it neurotoxicity by causing a barrage of insults, such as oxidative stress, mitochondrial apoptosis, inflammation, excitotoxicity, and formation of inclusion bodies acting singly and in concert, ultimately leading to dopaminergic neuronal damage in the substantia nigra pars compacta and striatum. The selective neurotoxicity induced by MPTP in the nigrostriatal dopaminergic neurons of the mouse brain has led to new perspectives on PD. For decades, the MPTP-induced mouse model of PD has been the gold standard in PD research even though it does not fully recapitulate PD symptomatology, but it does have the advantages of simplicity, practicability, affordability, and fewer ethical considerations and greater clinical correlation than those of other toxin models of PD. The model has rejuvenated PD research and opened new frontiers in the quest for more novel therapeutic and adjuvant agents for PD. Hence, this review summarizes the role of MPTP in producing Parkinson-like symptoms in mice and the experimental role of the MPTP-induced mouse model. We discussed recent developments of more promising PD therapeutics to enrich our existing knowledge about this neurotoxin using this model.
Citations
Downloads
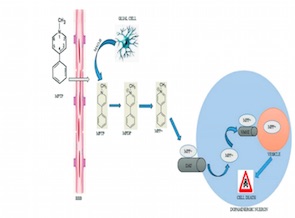
Downloads
Additional Files
Published
Issue
Section
Categories
How to Cite
Accepted 2020-12-10
Published 2021-08-01